
Cold-induced PA28γ/PSME-3 triggers trypsin-like activity
Given that exposure of C. elegans to low temperature (15 °C) after development is sufficient to induce lifespan extension9,12, we asked whether cold temperature influences proteasome activity during adulthood. To prevent the development of progeny, we used fer-15(b26);fem-1(hc17) mutant worms9,33, which are sterile when raised at the restrictive temperature (25 °C) during development. Similar to wild-type animals, cold temperature after development also extends lifespan in control sterile worms9. Thus, we raised control sterile worms at 25 °C until day 1 of adulthood and then shifted them to different temperatures. At day 6 of adulthood, worms exhibited a dramatic increase in trypsin-like proteasome activity at cold temperature (15 °C) when compared with 20 °C or 25 °C (Fig. 1a). However, adult control sterile worms had similar caspase-like and chymotrypsin-like proteasome activities across temperatures (Fig. 1b,c). The cold-induced effects on trypsin-like activity were not linked with sterility, as wild-type worms also had a similar increase when shifted from 20 °C to 15 °C during adulthood (Fig. 1d). In contrast, caspase-like and chymotrypsin-like activities remained similar or decreased in wild-type animals at cold temperature, respectively (Fig. 1e,f).
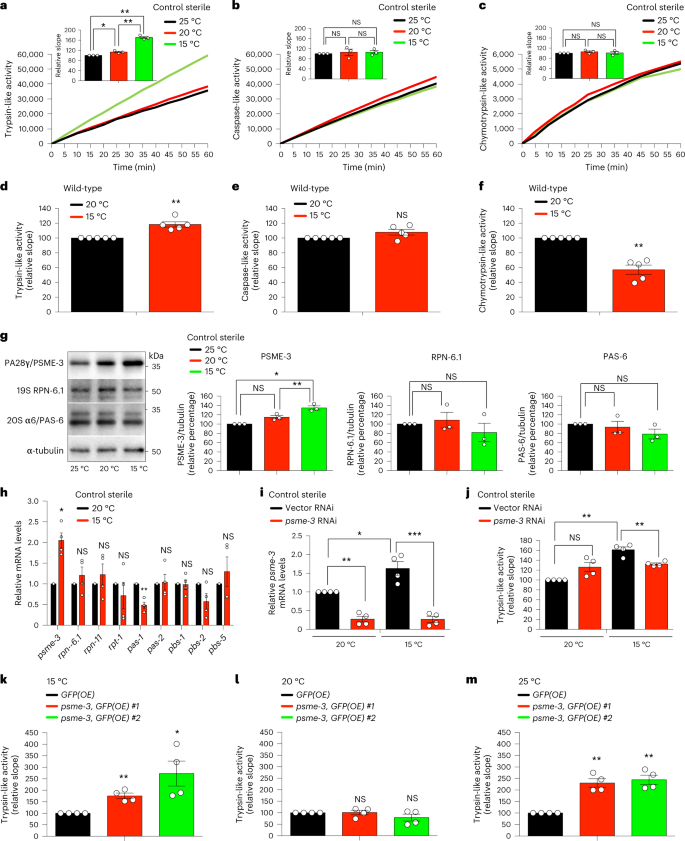
a–c, Trypsin-like (a), caspase-like (b) and chymotrypsin-like (c) proteasome activities in control sterile fer-15(b26);fem-1(hc17) C. elegans at day 6 of adulthood (mean ± standard error of the mean (s.e.m.) relative slope to 25 °C, n = 3 independent experiments). d–f, Trypsin-like (d), caspase-like (e) and chymotrypsin-like (f) proteasome activities in day 6 adult wild-type worms (mean ± s.e.m. relative slope to 20 °C, n = 5 independent experiments). g, Western blot of PA28γ/PSME-3, 19S RPN-6.1 and 20S α6/PAS-6 in day 6 adult control sterile worms. Graphs represent relative percentage values of proteasome subunits (corrected for α-tubulin loading control) to 25 °C (mean ± s.e.m., n = 3 independent experiments). h, mRNA levels in day 6 adult control sterile worms (mean ± s.e.m. relative expression to 20 °C, n = 4 independent experiments). i, Knockdown levels in day 6 adult control sterile worms on psme-3 RNAi initiated at day 1 of adulthood (mean ± s.e.m. relative expression to 20 °C vector RNAi, n = 4 independent experiments). j, Trypsin-like activity in day 6 adult control sterile worms on psme-3 knockdown (mean ± s.e.m. relative slope to 20 °C vector RNAi, n = 4 independent experiments). k, Somatic overexpression (OE) of psme-3 increases trypsin-like activity in adult worms at 15 °C (mean ± s.e.m. relative slope to control GFP(OE), n = 4 independent experiments). Two independent psme-3,GFP(OE) lines were tested. l, psme-3 overexpression does not increase trypsin-like activity at 20 °C (mean ± s.e.m. relative slope to control GFP(OE), n = 4 independent experiments). m, psme-3 overexpression increases trypsin-like activity at 25 °C (mean ± s.e.m. relative slope to control GFP(OE), n = 4 independent experiments). Control sterile worms were raised at 25 °C during development and then grown at the indicated temperatures until day 6 of adulthood. Wild-type and psme-3,GFP(OE) worms were raised at 20 °C during development and then grown at the indicated temperatures until day 6 of adulthood. Statistical comparisons were made by two-tailed Student’s t-test for paired samples. P value: *P < 0.05, **P < 0.01, ***P < 0.001, NS, not significant (P > 0.05). All the significant changes were also significant after correction for multiple testing by the false discovery rate (FDR) approach (FDR-adjusted P value (q value) < 0.05 was considered significant). Source Data contains exact P and q values.
Source data
To assess whether cold temperature changes the levels of proteasome subunits, we used available quantitative proteomics data9. We did not find substantial differences in 20S subunits comparing adult worms at cold (15 °C) and standard (20 °C) temperatures, including β2/PBS-2 that has trypsin-like activity (Extended Data Fig. 1a). Moreover, proteomic analysis did not detect significant changes in 19S subunits. Among PA28 subunits, C. elegans only expresses psme-3, the orthologue of human PA28γ/PSME3 (refs. 34,35). Notably, quantitative proteomics revealed increased levels of PSME-3 at cold temperature (Extended Data Fig. 1a). By western blot, we confirmed that worms exhibit higher protein levels of PSME-3 at 15 °C when compared with 20 °C or 25 °C (Fig. 1g). The increase in PSME-3 protein levels correlated with an upregulation of the mRNA amounts at cold temperature (Fig. 1h). Because PA28γ/PSME3 selectively activates trypsin-like activity in vitro31,36, we asked whether PSME-3 is required for the elevated trypsin-like activity at 15 °C. Indeed, knockdown of psme-3 after development decreased trypsin-like activity at cold temperature, but not at standard temperature (Extended Data Fig. 1b and Fig. 1i,j).
Because low temperature extends lifespan, we could not discard that the changes observed in day 6 adults ensue from differences in aging rates rather than a direct effect of PSME-3. To disentangle these possibilities, we examined worms at a younger age (that is, day 3 of adulthood). Importantly, cold temperature after development was sufficient to induce trypsin-like activity in both control sterile and wild-type animals at day 3 of adulthood (Extended Data Fig. 1c,d). Similar to day 6 adult worms, knockdown of psme-3 specifically blocked cold-induced trypsin-like activity in day 3 adults but had no effects at 20 °C (Extended Data Fig. 1c,d). To assess younger ages, we started the cold temperature and RNAi treatments during development and assessed proteasome activity at day 1 of adulthood. Indeed, day 1 adults exhibited higher levels of trypsin-like activity at 15 °C when compared with age-matched worms at 20 °C (Extended Data Fig. 1e). Although knockdown of psme-3 during development slightly decreased basal trypsin-like activity in day 1 adults at 20 °C, its inhibitory effects were much stronger at cold temperature (Extended Data Fig. 1e). Thus, these data support a direct effect of cold-induced PSME-3 in the upregulation of trypsin-like proteasome activity.
Along these lines, overexpression of psme-3 was sufficient to further increase trypsin-like activity at 15 °C (Fig. 1k). In contrast, psme-3 overexpression did not induce trypsin-like activity at 20 °C (Fig. 1l), suggesting that PSME-3 function requires its activation by other factors that occurs upon cold temperature. Additionally, worms may also have mechanisms to inactivate PSME-3 at 20 °C. However, worms did not inhibit overexpressed PSME-3 when subjected to mild heat stress, resulting in a pronounced increase of trypsin-like activity at 25 °C (Fig. 1m). Altogether, we found that elevated PA28γ/PSME-3 function underlies the high levels of trypsin-like activity induced by cold temperature in C. elegans, while overexpression of PSME-3 can also promote trypsin-like proteasome activity at warm temperature, but not standard temperature.
TRPA-1 is required for cold-induced trypsin-like activity
In C. elegans, low temperature activates the cold-sensitive channel TRPA-1, which promotes lifespan extension11,12. We found that mutant worms lacking trpa-1 have lower trypsin-like activity when compared with wild-type animals at cold temperature, either at day 3 or 6 of adulthood (Fig. 2a and Extended Data Fig. 1f). In contrast, loss of trpa-1 did not reduce basal trypsin-like activity at standard temperature (Extended Data Fig. 1g). Moreover, lack of trpa-1 did not affect a different proteasome activity at 15 °C, supporting that activation of TRPA-1 channels contributes to the selective induction of trypsin-like activity at low temperature (Extended Data Fig. 1h). Because loss of trpa-1 did not further decrease the low trypsin-like activity of psme-3 RNAi-treated worms at 15 °C, these results indicate that TRPA-1 channels modulate proteasome activity through PSME-3 (Fig. 2a).
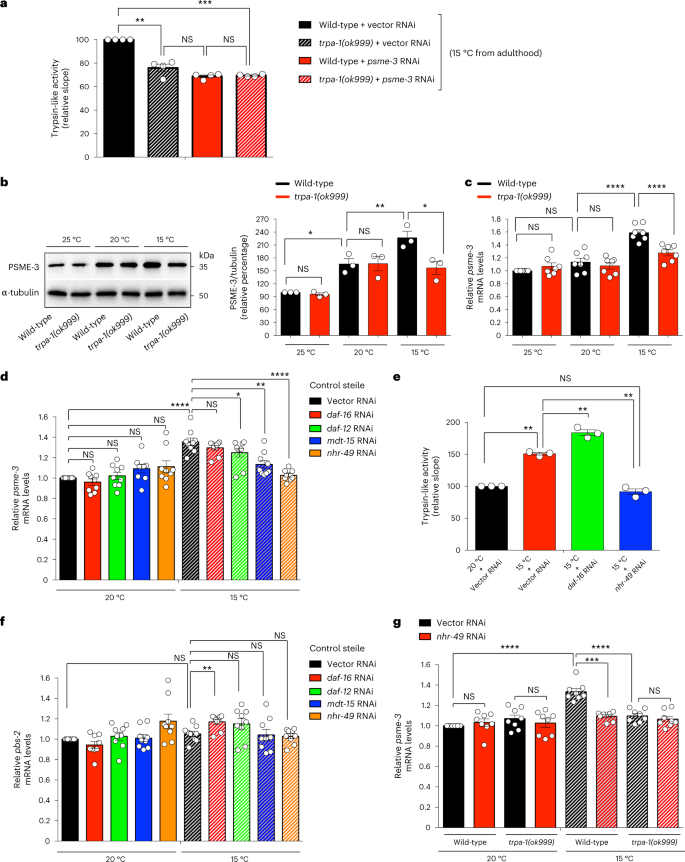
a, Trypsin-like proteasome activity in day 3-adult wild-type and trpa-1(ok999) mutant worms (mean ± s.e.m. relative slope to 15 °C vector RNAi, n = 4 independent experiments). b, Western blot of PA28γ/PSME-3 in day 6 adult wild-type and trpa-1(ok999) mutant worms. Graph represents the relative percentage values of PSME-3 (corrected for α-tubulin loading control) to 25 °C wild-type (mean ± s.e.m., n = 3 independent experiments). c, qPCR analysis of psme-3 mRNA levels in day 6 adult wild-type and trpa-1(ok999) mutant worms. Graph (relative expression to 25 °C wild-type) represents the mean ± s.e.m. of seven independent experiments. d, psme-3 mRNA levels in day 6 adult control sterile worms upon knockdown of distinct transcriptional regulators involved in cold-induced longevity. Graph (relative expression to 20 °C vector RNAi) represents the mean ± s.e.m. of 9 independent experiments. e, Knockdown of nhr-49 decreases cold-induced trypsin-like proteasome activity in control sterile worms (mean ± s.e.m. relative slope to 20 °C vector RNAi, n = 3 independent experiments). f, pbs-2 mRNA levels in day 6 adult control sterile worms. Graph (relative expression to 20 °C Vector RNAi) represents the mean ± s.e.m. of 9 independent experiments. g, psme-3 mRNA levels in day 6 adult wild-type and trpa-1(ok999) mutant worms. Graph (relative expression to 20 °C Vector RNAi) represents the mean ± s.e.m. of 8 independent experiments. In all the experiments, worms were raised at 20 °C until day 1 of adulthood and then grown at the indicated temperatures until day 3 (a) or 6 (b–g) of adulthood. Statistical comparisons were made by two-tailed Student’s t-test for paired samples. P value: *P < 0.05, **P < 0.01, ***P < 0.001, ****P < 0.0001; NS, P > 0.05. All the significant changes were also significant after correction for multiple testing by FDR approach (q value < 0.05). Source Data contains exact P and q values.
Source data
Indeed, we observed that lack of trpa-1 reduces the upregulation of PSME-3 protein levels at low temperature (Fig. 2b). Likewise, loss of trpa-1 also attenuated the induction of psme-3 mRNA amounts at 15 °C, suggesting that TRPA-1 modulates PSME-3 at the transcriptional level (Fig. 2c). As a sensor of cold temperature, TRPA-1 cannot promote transcriptional expression on its own and requires the activation of downstream transcription factors such as DAF-16/FOXO11. However, DAF-16 was not required for induction of psme-3 expression and trypsin-like activity (Fig. 2d,e). Besides DAF-16, other transcription factors are also involved in cold-induced longevity3. Among them, knockdown of the nuclear receptor daf-1223 partially reduced cold-induced psme-3 levels (Fig. 2d). However, we found the strongest inhibitory effects upon loss of the nuclear hormone receptor-49 (NHR-49), another transcription factor that promotes longevity at 15 °C22. Likewise, knockdown of the NHR-49 coregulator mdt-15/MED15 suppressed upregulation of psme-3 expression at 15 °C, but it did not affect psme-3 levels at 20 °C (Fig. 2d). Subsequently, loss of nhr-39 prevented cold-induced trypsin-like proteasome activity (Fig. 2e). Knockdown of nhr-49 did not affect the expression of the catalytic proteasome subunit pbs-2 (Fig. 2f), further supporting that nhr-49 induces trypsin-like activity through transcriptional regulation of psme-3. Notably, loss of nhr-49 did not further decrease psme-3 expression in mutant worms lacking trpa-1 (Fig. 2g). Thus, TRPA-1 channels induce psme-3 levels at cold temperature through NHR-49 transcription factor.
PA28γ/PSME-3 promotes cold-induced longevity
Notably, knockdown of PA28γ/psme-3 after development reduced the long lifespan phenotype induced by cold temperature (15 °C), but it did not shorten lifespan at either 20 °C or 25 °C (Fig. 3a–c). In contrast, knockdown of rpn-6.1, a specific activator of 26S proteasomes33,37, shortened lifespan at all the temperatures tested (Fig. 3d–f). These data indicate that 26S proteasomes are essential for viability at different temperatures33,38, whereas PA28γ/PSME-3 is particularly required for cold-induced longevity.
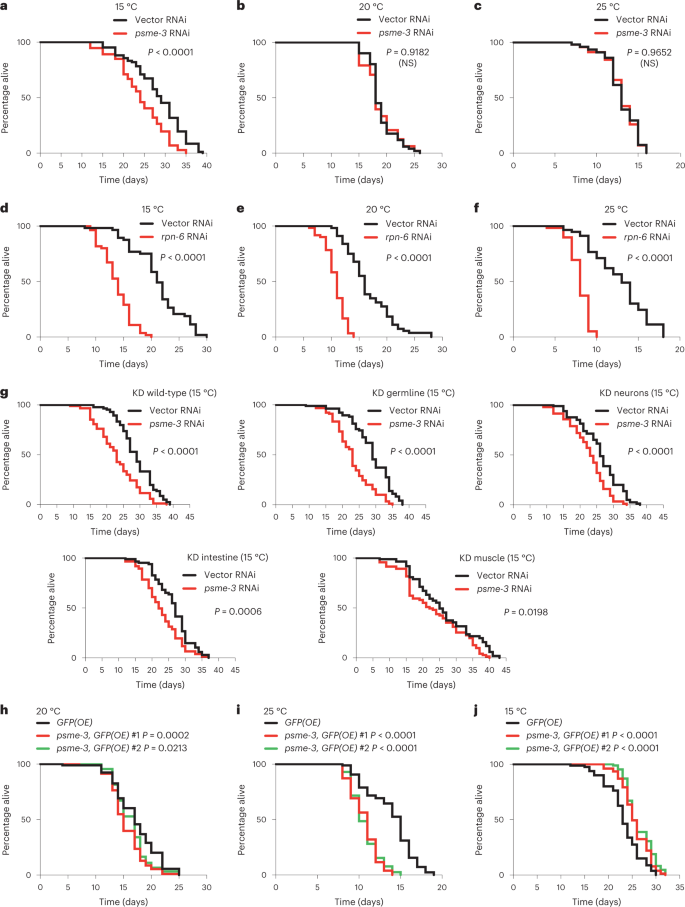
a, Knockdown of psme-3 shortens cold-induced longevity (15 °C) in wild-type worms. Vector RNAi mean ± s.e.m.: 28.26 days ± 0.69, psme-3 RNAi: 24.19 ± 0.67. b, psme-3 RNAi does not reduce the lifespan of wild-type worms at 20 °C. Vector RNAi mean ± s.e.m.: 18.92 ± 0.34, psme-3 RNAi: 18.71 ± 0.42. c, psme-3 RNAi does not further shorten the lifespan of wild-type worms at 25 °C. Vector RNAi mean ± s.e.m.: 13.03 ± 0.30, psme-3 RNAi: 13.02 ± 0.30. d, rpn-6.1 RNAi shortens lifespan of wild-type worms at 15 °C. Vector RNAi mean ± s.e.m.: 21.13 ± 0.62, rpn-6.1 RNAi: 13.73 ± 0.36. e, rpn-6.1 RNAi shortens lifespan of wild-type worms at 20 °C. Vector RNAi mean ± s.e.m.: 16.60 ± 0.55, rpn-6.1 RNAi: 10.72 ± 0.23. f, rpn-6.1 RNAi shortens lifespan of wild-type worms at 25 °C. Vector RNAi mean ± s.e.m.: 12.75 ± 0.44, rpn-6.1 RNAi: 7.98 ± 0.15. In panels a–f, RNAi was initiated at day 1 of adulthood because rpn-6.1 is required for larval development. g, Lifespan at 15 °C upon psme-3 RNAi treatment in wild-type animals (vector RNAi mean ± s.e.m.: 28.83 ± 0.58, psme-3 RNAi: 23.03 ± 0.67) or RNAi-deficient animals in which RNAi efficiency has been rescued in specific tissues. Tissue-specific knockdown (KD) of psme-3 in the germline (vector RNAi mean ± s.e.m.: 28.84 ± 0.68, psme-3 RNAi: 23.04 ± 0.58), neurons (vector RNAi: 26.04 ± 0.67, psme-3 RNAi: 22.56 ± 0.59), intestine (vector RNAi: 26.37 ± 0.62, psme-3 RNAi: 22.85 ± 0.62) or muscle (vector RNAi: 26.35 ± 1.21, psme-3 RNAi: 23.37 ± 1.05) shortens cold-induced longevity at 15 °C. Knockdown was initiated from hatching. h, Somatic overexpression of psme-3 under the sur-5 promoter slightly shortens lifespan at 20 °C. GFP(OE) mean ± s.e.m.: 17.19 ± 0.41, psme-3,GFP(OE) #1: 15.48 ± 0.31, psme-3,GFP(OE) #2: 16.31 ± 0.31. i, Somatic overexpression of psme-3 is deleterious for adult lifespan at 25 °C. GFP(OE) mean ± s.e.m.: 13.83 ± 0.31, psme-3,GFP(OE) #1: 10.60 ± 0.19, psme-3,GFP(OE) #2: 10.67 ± 0.19. j, Overexpression of psme-3 in somatic tissues extends lifespan at 15 °C. GFP(OE) mean ± s.e.m.: 23.26 ± 0.43, psme-3,GFP(OE) #1: 26.65 ± 0.33, psme-3,GFP(OE) #2: 26.38 ± 0.31. Worms were raised at 20 °C and shifted to the indicated temperatures after development. In all the experiments, P values were calculated by two-sided log-rank test, n = 96 worms per condition. NS, P > 0.05). Supplementary Table 3 contains statistical analysis and replicate data of independent lifespan experiments.
Intrigued by these findings, we asked in which tissues PSME-3 acts to regulate organismal lifespan. Although TRPA-1 is expressed in multiple tissues, its activation in the intestine and neurons is particularly important to promote longevity11. However, activation of TRPA-1 in neurons also signals distal tissues such as the germline to delay reproductive aging9. In turn, the germline releases factors to induce pro-longevity genes in somatic tissues, including the intestine and muscle9. According to its key role in cell non-autonomous regulation of cold-induced longevity9, knockdown of psme-3 in the germline alone resulted in the strongest decrease of lifespan at 15 °C (Fig. 3g). In addition, tissue-specific knockdown of psme-3 in neurons, intestine or muscle also significantly decreased lifespan at 15 °C (Fig. 3g). Thus, PSME-3 has a pro-longevity role in all the tissues which are known to influence cold-induced lifespan extension (that is germline, neurons, intestine and muscle). To assess whether PSME-3 is expressed in these tissues, we tagged endogenous PSME-3 protein with GFP. We found that worms exhibit a robust basal expression of PSME-3 across tissues, even at standard temperature (Extended Data Fig. 2a). Because cold temperature only induces a moderate increase in PSME-3 levels (Fig. 1g), we could not use the reporter strain to assess whether PSME-3 is upregulated in a particular tissue at 15 °C (Extended Data Fig. 2a–c). Nevertheless, we confirmed that PSME-3 is highly expressed not only in the germline and intestine but also in muscle and neurons, although to a lesser extent (Extended Data Fig. 2a–d). Importantly, specific knockdown of psme-3 in the aforementioned tissues decreased trypsin-like activity (Extended Data Fig. 2e–h), further supporting a functional role of PSME-3 in the germline, intestine, muscle and neurons.
We then assessed whether PSME-3 overexpression in somatic tissues is sufficient to promote longevity. Interestingly, PA28γ/psme-3 overexpression slightly decreased lifespan at standard temperature (20 °C) and was strongly detrimental at warmer temperature (Fig. 3h,i). On the contrary, PA28γ/psme-3 overexpression further extends longevity at 15 °C (Fig. 3j). Whereas moderate cold temperature (15 °C) can be advantageous and prolongs lifespan, extreme low temperatures (for example 4 °C) are harmful for C. elegans39. To examine whether PA28γ/PSME-3 can confer resistance to acute cold shock, we exposed worms to extreme low temperature (4 °C) for 12 h and then shifted them back to 20 °C. We found that overexpression of PA28γ/psme-3 did not increase survival after exposure to acute cold shock (Extended Data Fig. 3a). Thus, our data indicate that PA28γ/PSME-3 does not protect from extreme low temperatures but contributes to the longevity effects induced by moderate cold temperature. However, PSME-3 overexpression can have negative effects on lifespan at higher temperatures.
Given that activation of TRPA-1 promotes cold-induced longevity, we performed epistasis experiments to assess its functional links with PSME-3 in lifespan regulation. Similar to wild-type animals, knockdown of psme-3 did not shorten lifespan of trpa-1-lacking worms at standard (20 °C) and warmer (25 °C) temperatures (Extended Data Fig. 3b,c). Whereas loss of psme-3 shortened the cold-induced long lifespan of wild-type animals, it did not further decrease the short lifespan of trpa-1 mutant worms at cold temperature (15 °C) (Extended Data Fig. 3d). Moreover, overexpression of psme-3 also shortened the lifespan of trpa-1 mutant animals at both standard and warm temperatures, but not at cold temperature (Extended Data Fig. 3e–g). Although psme-3 overexpression slightly increased the mean lifespan of trpa-1 mutant worms at 15 °C, this extension was not significant (Extended Data Fig. 3g). Together, our results suggest that TRPA-1 acts upstream of PSME-3 and is required for its longevity effects in C. elegans at moderate low temperature.
Low temperature prevents disease-related protein aggregation
In contrast to 26S proteasomes, activation of the 20S proteasome through PA28γ induces protein degradation in a ubiquitin-independent manner31. Aging triggers a global loss of ubiquitination through the proteome in C. elegans, reducing the degradation of multiple proteins by the 26S proteasome26. Subsequently, these dysregulated proteasome targets accumulate during aging and impair cellular function26. For instance, the intermediate filament IFB-2 escapes the clean-up by the 26S proteasome with age, leading to its aggregation within intestinal cells. Conversely, knockdown of IFB-2 during adulthood delays the decline in intestinal integrity characteristic of aging animals and extends lifespan at standard temperature26. Notably, we found that cold temperature prevents the accumulation of IFB-2 and its subsequent aggregation in adult wild-type animals with age (Extended Data Fig. 4a,b). However, knockdown of PA28γ/psme-3 reduces the degradation of IFB-2, suppressing the ameliorative effects of low temperature on IFB-2 aggregation (Extended Data Fig. 4c,d). Thus, these results suggest that cold-induced PA28γ/PSME-3 can ameliorate age-related deficits in protein degradation.
In vitro, PA28γ preferentially promotes the degradation of unstructured proteins over their native/folded counterparts, including unfolded variants of β-casein and insulin-like growth factor 1 (IGF-1)40. Moreover, in vitro experiments demonstrated that PA28γ promotes the degradation of peptides containing expanded polyglutamine repeats (polyQ)41, which are linked with different human diseases. Thus, we asked whether cold-induced PA28γ/PSME-3 modulates the levels of disease-related mutant proteins which are prone to misfolding and aggregation. To this end, we used C. elegans that specifically express expanded polyQ peptides in neurons. These worms replicate key features of Huntington’s disease, as protein aggregation and neurotoxicity correlate with increased length of the polyQ peptide, with a pathogenic threshold of 40 repeats42,43,44,45. By filter trap assay, we did not observe aggregation of control polyQ19 peptides at any of the tested temperatures. In contrast, polyQ67-expressing worms had a strong aggregation phenotype at standard temperature (20 °C) which was further increased by mild heat-stress (25 °C) (Fig. 4a). Notably, cold temperature (15 °C) attenuated polyQ67 aggregation when compared with standard temperature, correlating with a downregulation in the total amounts of polyQ67 protein (Fig. 4a,b). On the contrary, low temperature did not decrease the protein levels of control polyQ19 when compared with standard temperature (Fig. 4b).
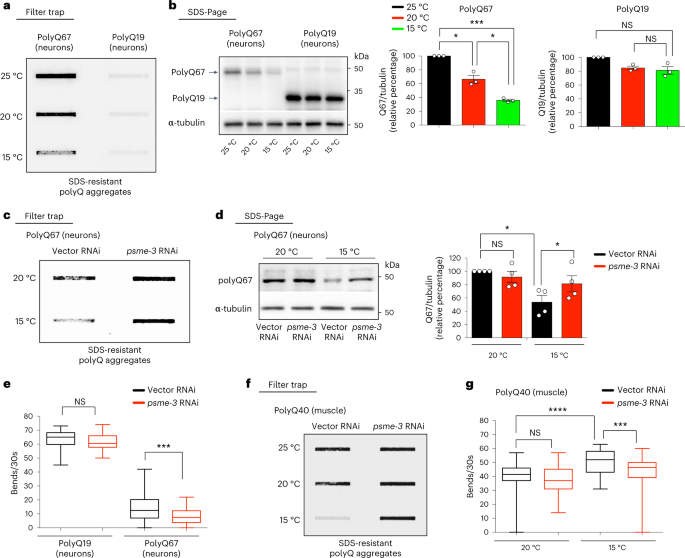
a, Filter trap analysis of day 6 adult worms that express polyQ67::YFP or control polyQ19::CFP (detected by anti-GFP antibody) in neurons. Representative of three independent experiments. b, Western blot of day 6 adult worms to assess total polyQ67::YFP and polyQ19::CFP levels (detected by anti-GFP antibody). Graphs represent the relative percentage values of polyQ67 and polyQ19 protein levels (corrected for α-tubulin loading control) to 25 °C (mean ± s.e.m., n = 3 independent experiments). c, Filter trap of polyQ67::YFP aggregation upon psme-3 RNAi at the indicated temperatures. Worms were analyzed at day 6 of adulthood. Representative of three independent experiments. d, Western blot of total polyQ67 protein levels on psme-3 RNAi. Graph represents the relative percentage of polyQ67 protein levels (corrected for α-tubulin loading control) to 20 °C Vector RNAi (mean ± s.e.m., n = 4 independent experiments). Worms were analyzed at day 6 of adulthood. e, Thrashing movements over a 30-s period at day 3 of adulthood (n = 50 worms per condition from three independent experiments). The box plot represents the 25th–75th percentiles, the line depicts the median and the whiskers show the min–max values. f, Filter trap analysis of C. elegans that express polyQ40::YFP in the muscle alone (detected by anti-GFP antibody). Worms were analyzed at day 6 of adulthood. Representative of four independent experiments. g, Thrashing movements over a 30-s period at day 3 of adulthood (n = 50 worms per condition from three independent experiments). The box plot represents the 25th–75th percentiles, the line depicts the median and the whiskers show the min–max values. In all the experiments, worms were shifted at the indicated temperatures after development and RNAi was initiated at day 1 of adulthood. Statistical comparisons were made by two-tailed Student’s t-test for paired (b,d) or unpaired samples (e,g). P value: *P < 0.05, ***P < 0.001, ****P < 0.0001; NS, P > 0.05). All the significant changes were also significant after correction for multiple testing by FDR approach (q value < 0.05). Source Data contains for exact P and q values.
Source data
Knockdown of PA28γ/psme-3 diminished the cold-induced degradation of polyQ67, increasing its aggregation at 15 °C (Fig. 4c,d). The aggregation of polyQ67 in neurons causes neurotoxicity and subsequently impairs motility, a disease-like phenotype42,43. We found that loss of PA28γ/psme-3 reduces the motility of neuronal polyQ67-expressing worms at cold temperature, but does not affect control polyQ19 worms (Fig. 4e). We then asked whether PSME-3 influences neuronal aggregation of polyQ67 through its intracellular activity in neurons or via cell non-autonomous effects triggered by its function in other tissues. Notably, neuronal-specific knockdown of psme-3 was sufficient to induce aggregation of polyQ67 protein in neurons (Extended Data Fig. 5a). Thus, these results indicate that intracellular levels of PSME-3 can directly regulate proteostasis of polyQ-expanded proteins in neurons. Because overexpression of psme-3 induces trypsin-like activity at both 15 °C and 25 °C (Fig. 1k,m), we assessed the effects of psme-3 overexpression in polyQ67-expressing worms. We found that psme-3 overexpression reduces the total levels of neuronal polyQ67 at both cold and warm temperatures (Extended Data Fig. 5b,c). Concomitantly, psme-3 overexpression diminished the accumulation of aggregated polyQ67 peptides as well as motility defects at 25 °C (Extended Data Fig. 5d,e). Given the low levels of aggregated polyQ67 at cold temperature, we could not detect a further decrease upon psme-3 overexpression at 15 °C (Extended Data Fig. 5d). Nevertheless, psme-3 overexpression was sufficient to further ameliorate motility defects at 15 °C (Extended Data Fig. 5e). In contrast, psme-3 overexpression did not influence the levels, aggregation and neurotoxicity of polyQ67 at 20 °C (Extended Data Fig. 5f–h), according to its lack of effects on proteasome activity at standard temperature (Fig. 1l).
In addition to neurons, low temperature also decreased polyQ levels and aggregation in C. elegans that specifically express expanded polyQ peptides in the muscle (Fig. 4f and Extended Data Fig. 5i). Moreover, knockdown of PA28γ/psme-3 reduced the degradation of muscle polyQ peptides (Extended Data Fig. 5j). Conversely, loss of psme-3 suppressed the inhibitory effects of cold temperature over polyQ aggregation in the muscle (Fig. 4f). The aggregation of expanded polyQ within muscle cells has intracellular detrimental effects in C. elegans, reducing muscle function and organismal motility46. In correlation with polyQ aggregates levels in the muscle, cold temperature ameliorated the deficits in coordinated movement whereas loss of psme-3 suppressed these beneficial effects (Fig. 4g). Therefore, cold-induced PA28γ/PSME-3 can prevent the aggregation of expanded polyQ proteins in distinct tissues, alleviating their pathological effects.
Besides expanded polyQ proteins, we asked whether cold-induced PA28γ/PSME-3 can also diminish aggregation of other disease-related proteins. To this end, we examined C. elegans that express an ALS-related mutant variant of FUS protein (FUSP525L) in neurons47. These worms replicate pathological phenotypes of ALS such as protein aggregation and neurodegeneration, as reflected by loss of motility43,47. We found that cold temperature decreases the total levels of mutant FUS protein, resulting in lower disease-related aggregation compared to either 20 °C or 25 °C (Fig. 5a,b). However, knockdown of PA28γ/psme-3 diminished the cold-induced degradation of mutant FUS, triggering the accumulation of FUS aggregates at 15 °C (Fig. 5c,d). Subsequently, knockdown of psme-3 suppressed the beneficial effects of cold temperature on ALS-related motility deficits (Fig. 5e). In addition to mutant FUS, cold temperature also decreased the protein levels and aggregation of ALS-related TDP-43M331V variant (Fig. 5f,g), another mutant protein which is prone to aggregation48. Conversely, knockdown of PA28γ/psme-3 reduced the cold-induced degradation of mutant TDP-43, promoting its aggregation at 15 °C (Fig. 5h,i). Collectively, our data indicate that cold-induced PA28γ/PSME-3 can attenuate the pathological aggregation of distinct disease-related proteins in C. elegans models.
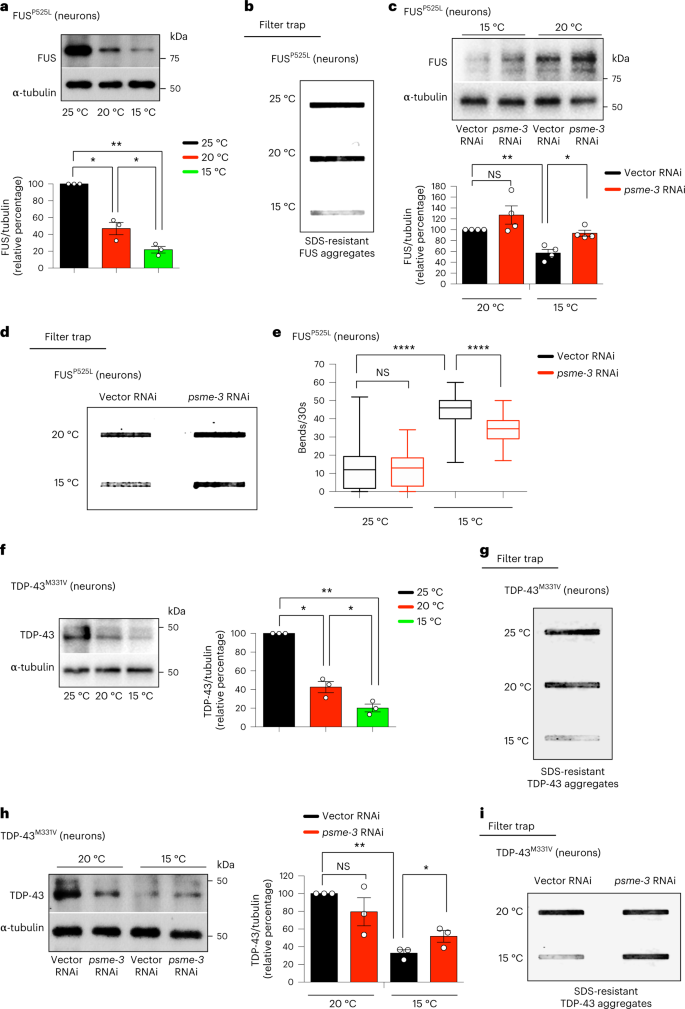
a, Western blot with anti-FUS antibody of day 6 adult worms expressing ALS-related FUSP525L mutant variant in neurons. Graph represents the relative percentage of FUSP525L protein levels (corrected for α-tubulin loading control) to 25 °C (mean ± s.e.m., n = 3 independent experiments). b, Cold temperature decreases FUSP525L aggregation in day 6 adult worms (detected by filter trap with anti-FUS antibody). Representative of three independent experiments. c, Western blot of FUSP525L levels upon psme-3 RNAi at different temperatures. Graph represents the relative percentage of FUSP525L levels (corrected for α-tubulin) to 20 °C vector RNAi (mean ± s.e.m., n = 4 independent experiments). d, Filter trap of FUSP525L aggregation upon psme-3 RNAi in day 6 adult worms. Representative of five independent experiments. e, Thrashing movements over a 30-s period at day 3 of adulthood (n = 50 worms from three independent experiments). The box plot represents the 25th–75th percentiles, the line depicts the median and the whiskers show the min–max values. f, Western blot with anti-TDP-43 antibody of day 6 adult worms expressing ALS-related TDP-43M331V mutant variant in neurons. Graph represents the relative percentage of TDP-43M331V protein levels (corrected for α-tubulin) to 25 °C (mean ± s.e.m., n = 3 independent experiments). g, Cold temperature decreases TDP-43M331V aggregation in day 6 adult worms (detected by filter trap with anti-TDP-43 antibody). Representative of three independent experiments. h, Western blot of TDP-43M331V levels upon knockdown of PA28γ/psme-3 at different temperatures. Graph represents the relative percentage of TDP-43M331V levels (corrected for α-tubulin) to 20 °C Vector RNAi (mean ± s.e.m., n = 3 independent experiments). i, Filter trap analysis of TDP-43M331V aggregation upon psme-3 RNAi in day 6 adult worms. Representative of three independent experiments. In all the experiments, worms were shifted to the indicated temperatures after development and RNAi was initiated after development. Statistical comparisons were made by two-tailed Student’s t-test for paired (a,c,f,h) or unpaired samples (e). P value: *P < 0.05, **P < 0.01, ****P < 0.0001; NS, P > 0.05). Significant changes were also significant after correction for multiple testing by FDR (q value < 0.05). Source Data contains for exact P and q values.
Source data
Cold temperature induces PA28γ-proteasomes in human cells
The human body can physiologically reach moderate cold temperatures (36 °C) during sleep, but a pathological acute drop in temperature (≤ 35 °C) leads to hypothermia19. To assess whether a moderate cooling also influences proteasome activity in human cells, we shifted HEK293 human cells from the standard temperature (37 °C) to moderate cold temperature (36 °C) for 24 h. Similar to C. elegans, moderate cold temperature increased trypsin-like activity in human cells, whereas the other two activities of the proteasome remained similar (Fig. 6a–c). However, a further decrease in temperature to 35 °C not only downregulated trypsin-like activity but also caspase-like activity when compared with 37 °C (Extended Data Fig. 6a,b). Thus, these results indicate that moderate cold temperature (36 °C) can selectively induce trypsin-like activity in human cells, but lower temperatures can have a general negative effect on proteasome activities. Because HEK293 cells expressed endogenous levels of TRPA1 (Extended Data Fig. 6c,d), we asked whether this cold-sensitive channel also regulates the induction of trypsin-like activity in human cells at moderate cold temperature. Indeed, either knockdown of TRPA1 or treatment with HC-030031, a selective antagonist of TRPA149,50, blocked the trypsin-like activity induced by cold temperature in HEK293 cells (Extended Data Fig. 6e,f and Fig. 6d).
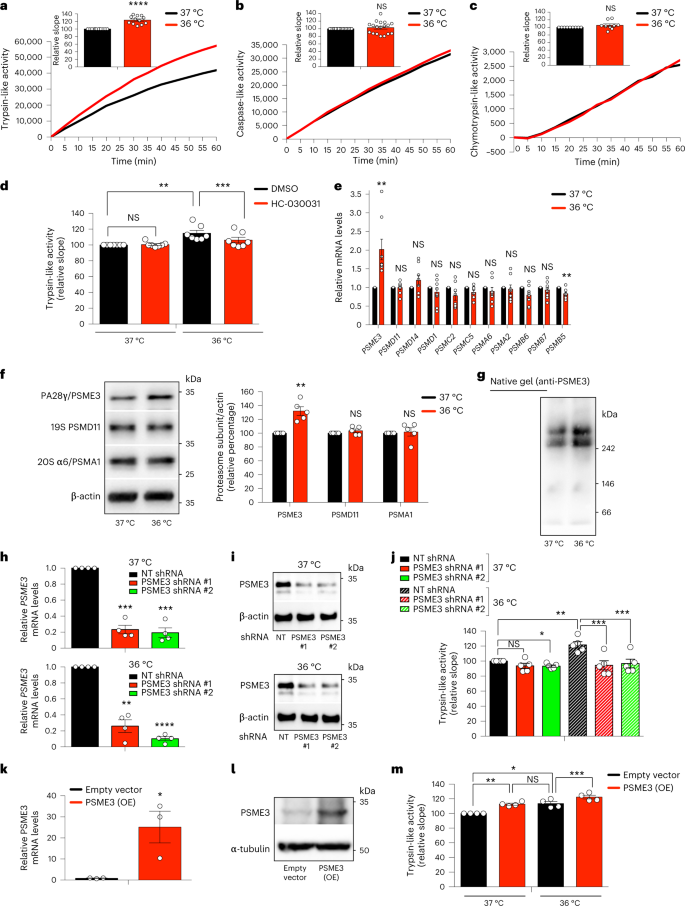
a, Trypsin-like activity in HEK293 cells at cold temperature (36 °C) for 24 h (mean ± s.e.m. relative slope to 37 °C, n = 12 independent experiments). b, Caspase-like activity in HEK293 cells (mean ± s.e.m. relative to 37 °C, n = 18 independent experiments). c, Chymotrypsin-like activity in HEK293 cells (mean ± s.e.m. relative to 37 °C, n = 9 independent experiments). d, Trypsin-like activity in HEK293 cells upon 25 µM HC-030031 for 24 h (mean ± s.e.m. relative to 37 °C + DMSO vehicle control, n = 7 independent experiments). e, mRNA levels of proteasome subunits in HEK293 cells (mean ± s.e.m. relative expression to 37 °C, n = 8 independent experiments). f, Western blot of PA28γ/PSME3, 19S PSMD11, and 20S α6/PSMA1 in HEK293 cells. Graph represents relative percentage values of proteasome subunits (corrected for β-actin loading control) to 37 °C (mean ± s.e.m., n = 5 independent experiments). g, Native gel electrophoresis of HEK293 cells followed by immunoblotting with anti-PSME3 antibody. Representative of 5 independent experiments. h, Knockdown levels in HEK293 cells expressing PSME3 shRNA (mean ± s.e.m. relative to non-targeting (NT) shRNA, n = 4 independent experiments). i, PSME3 protein levels in PSME3 shRNA-HEK293 cells. β-actin loading control. Representative of three independent experiments. j, Trypsin-like activity in PSME3-shRNA-HEK293 cells (mean ± s.e.m. relative to 37 °C NT shRNA, n = 5 independent experiments). k, PSME3 mRNA levels in HEK293 cells overexpressing (OE) PSME3 at 37 °C (mean ± s.e.m. relative to empty vector, n = 3 biological replicates). l, PSME3 protein levels in PSME3(OE)-HEK293 cells at 37 °C. α-tubulin loading control. Representative of four independent experiments. m, PSME3 overexpression increases trypsin-like activity at 37 °C and 36 °C (mean ± s.e.m. relative to 37 °C + empty vector, n = 4 independent experiments). In all the experiments, cells were cultured at 37 °C and then shifted to cold temperature (36 °C) or maintained at 37 °C for 24 h before the analysis. Statistical comparisons were made by two-tailed Student’s t-test for paired samples, except Fig. 6k (unpaired t-test). P value: *P < 0.05, **P < 0.01, ***P < 0.001, ****P < 0.0001, NS, P > 0.05). All the significant changes were also significant after correction for multiple testing by FDR (q value < 0.05). Source Data contains exact P and q values.
Source data
Moderate cold temperature (36 °C) resulted in increased transcript and protein levels of PSME3, the human orthologue of PA28γ (Fig. 6e,f). Moreover, we found increased assembly of PSME3 subunits into proteasome-activator 11S/PA28γ7 complexes at 36 °C (Fig. 6g). To further determine whether PSME3 underlies the upregulation of trypsin-like activity triggered by moderate cold temperature, we generated stable PSME3-shRNA expressing HEK293 lines. These cells exhibited robust knockdown of PSME3 at either standard or cold temperatures (Fig. 6h,i). Remarkably, loss of PSME3 prevented the upregulation of trypsin-like activity induced by moderate cold temperature, but did not have strong effects at standard temperature (Fig. 6j). Moreover, PSME3 overexpression further increased trypsin-like activity at moderate cold temperature (Fig. 6k-m). In contrast to C. elegans, PSME3 overexpression was also sufficient to induce trypsin-like activity at 37 °C (Fig. 6k-m), raising the possibility that PSME3 can have valuable effects in human cells even at normal temperature.
PA28γ-proteasomes degrade human disease-related proteins
Given the beneficial impact of cold temperature in C. elegans, we asked whether low temperature also prevents disease-related protein aggregation in human cells. To this end, we generated HEK293 cell models that express either control (Q23) or polyQ-expanded (Q100) huntingtin (HTT), the mutant protein underlying Huntington’s disease51,52. In these cells, expression of mutant Q100-HTT resulted in the accumulation of polyQ aggregates, whereas control Q23-HTT did not form aggregates (Fig. 7a,b). Moderate cold temperature (36 °C) substantially reduced the amounts of Q100-HTT protein and its aggregation, but it did not influence control Q23-HTT levels (Fig. 7a,b). We found that either the knockdown or inhibition of TRPA1 channels is sufficient to block the cold-induced degradation of mutant HTT, leading to its aggregation at 36 °C (Fig. 7c–f). Likewise, stable PSME3-knockdown HEK293 cells lost their cold-induced ability to promote degradation of mutant HTT, resulting in similar levels of polyQ-expanded aggregates at cold and standard temperatures (Fig. 7g,h). In contrast, loss of PSME3 did not further increase the protein levels and aggregation of mutant HTT at standard temperature (37 °C) (Fig. 7g,h). Moreover, knockdown of PSME3 did not affect control Q23-HTT levels at either standard or low temperature, supporting that cold-induced PA28γ/PSME3 preferentially promotes the degradation of mutant HTT (Fig. 7g,h). Given that PSME3 overexpression induces trypsin-like activity even at normal temperature, we assessed whether increasing PSME3 levels prevents mutant HTT aggregation at 37 °C. Indeed, PSME3 overexpression was sufficient to promote the degradation of mutant HTT, reducing its aggregation at normal temperature (Fig. 7i,j). Because the aggregated amounts of mutant HTT were very low at 36 °C, it was difficult to interpret whether PSME3 overexpression further decreases its aggregation at cold temperature (Fig. 7j).
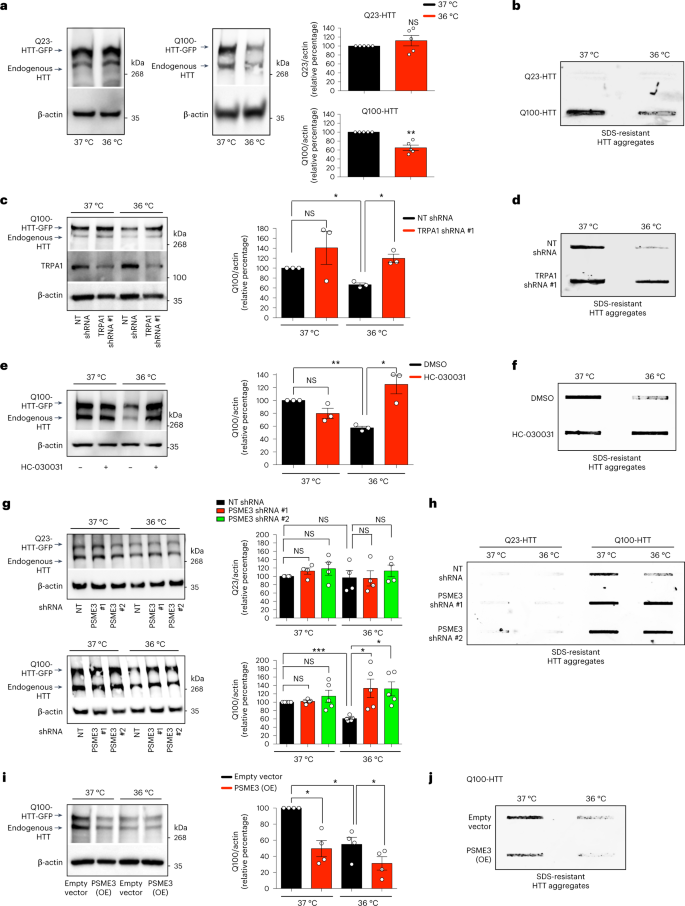
a, Western blot with anti-HTT antibody in HEK293 cells expressing control Q23-HTT-GFP or aggregation-prone Q100-HTT-GFP. Graphs: mean ± s.e.m. relative percentage of Q23-HTT or Q100-HTT levels (corrected for β-actin loading control) to 37 °C, n = 5 independent experiments. b, Filter trap with anti-GFP antibody of HEK293 cells expressing Q23-HTT-GFP or Q100-HTT-GFP. Representative of five independent experiments. c, Western blot with anti-HTT antibody in Q100-HTT-GFP HEK293 cells upon TRPA1 shRNA. Graph: mean ± s.e.m. relative percentage of Q100-HTT levels (corrected for β-actin) to 37 °C + non-targeting (NT) shRNA, n = 3 independent experiments. d, Filter trap with anti-GFP of Q100-HTT-GFP HEK293 cells upon TRPA1 shRNA. Representative of three independent experiments. e, Western blot with anti-HTT in Q100-HTT-GFP HEK293 cells treated with 25 µM HC-030031 (24 h). Graph: mean ± s.e.m. relative percentage of Q100-HTT levels (corrected for β-actin) to 37 °C + DMSO vehicle control, n = 3 independent experiments. f, Filter trap with anti-GFP of Q100-HTT-GFP aggregates in HEK293 cells treated with 25 µM HC-030031 (24 h). Representative of three independent experiments. g, Western blot with anti-HTT antibody in HEK293 cells expressing control Q23-HTT-GFP or Q100-HTT-GFP upon PSME3 shRNA. Graphs: mean ± s.e.m. relative percentage of Q23-HTT (n = 4) or Q100-HTT (n = 5) levels (corrected for β-actin) to the corresponding 37 °C + NT shRNA. h, Filter trap with anti-GFP of HEK293 cells expressing Q23-HTT-GFP or Q100-HTT-GFP upon PSME3 shRNA. Representative of six independent experiments. i, Western blot with anti-HTT of PSME3(OE)-HEK293 cells expressing Q100-HTT-GFP. Graph: mean ± s.e.m. relative percentage of Q100-HTT levels (corrected for β-actin) to 37 °C + Empty vector, n = 4 independent experiments. j, Filter trap with anti-GFP of PSME3(OE)-HEK293 cells expressing Q100-HTT-GFP. Representative of four independent experiments. In all the experiments, cells were cultured at 37 °C and then shifted to cold temperature (36 °C) or maintained at 37 °C for 24 h before the analysis. Comparisons were made by two-tailed Student’s t-test for paired samples. P value: *P < 0.05, **P < 0.01, ***P < 0.001; NS, P > 0.05). All the significant changes were also significant after correction for multiple testing by FDR (q value < 0.05). Source Data contains exact P and q values.
Source data
Besides polyQ-expanded HTT, cold temperature also diminished the levels and aggregation of the ALS-related mutant FUSP525L variant in HEK293 human cells (Fig. 8a,b). Knockdown of PSME3 suppressed the cold-induced degradation of aggregation-prone FUSP525L, resulting in the accumulation of FUS aggregates in HEK293 cells at cold temperature (Fig. 8a,b). In contrast, cold-induced PA28γ/PSME3 did not change wild-type FUS levels (Fig. 8a,b). Importantly, either knockdown or pharmacological inhibition of TRPA1 also triggered mutant FUS aggregation at cold temperature (Extended Data Fig. 7a–c). Because TRPA1 inhibition did not further increase mutant FUS aggregation in PSME3-knockdown HEK293 cells, these results further support a role of TRPA1 on PSME3 effects at cold temperature (Extended Data Fig. 7c). However, PSME3 overexpression could circumvent the requirement for TRPA1 and promote degradation of FUSP525L at 37 °C, reducing mutant FUS aggregates in human cells at normal temperature (Fig. 8c,d).
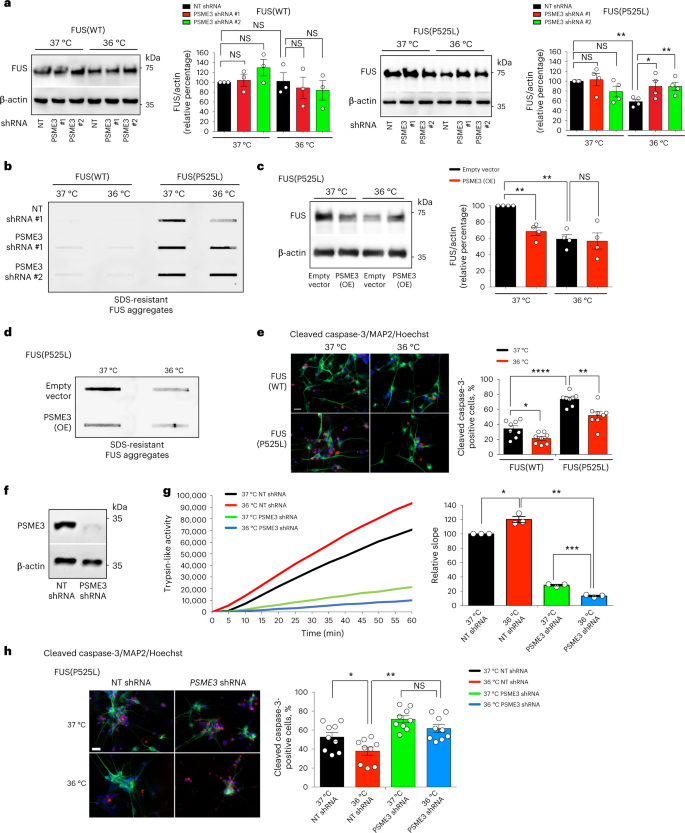
a, Western blot with anti-FUS antibody in HEK293 cells expressing wild-type FUS (WT) or ALS-related mutant FUSP525L. Graphs represent the relative percentage of FUS levels (corrected for β-actin loading control) to the corresponding 37 °C + non-targeting (NT) shRNA (mean ± s.e.m, FUS(WT): n = 3; FUS(P525L): n = 4). b, Filter trap with anti-FUS of HEK293 cells expressing wild-type or mutant FUS. Representative of five independent experiments. c, Western blot with anti-FUS of PSME3(OE)-HEK293 cells expressing mutant FUS. Graph represents the relative percentage of FUS levels (corrected for β-actin) to 37 °C + Empty vector (mean ± s.e.m. of 4 independent experiments). d, Filter trap with anti-FUS of PSME3(OE)-HEK293 cells expressing mutant FUS(P525L). Representative of four independent experiments. e, Immunocytochemistry of ALS (FUSP525L/P525L) and isogenic control (FUSWT/WT) iPSC-derived motor neurons. Cleaved caspase-3 (red), MAP2 (green) or Hoechst (blue) staining was used as a marker of apoptosis, neurons and nuclei, respectively. Scale bar: 20 µm. Graph represents the percentage of cleaved caspase-3-positive cells/total nuclei (mean ± s.e.m. of eight biological replicates, FUS(WT) 37 °C: 998 total nuclei; FUS(WT) 36 °C: 661; FUS(P525L) 37 °C: 331, FUS(P525L) 36 °C: 265). f, Western blot with anti-PSME3 of ALS-iPSCs expressing PSME3 shRNA. β-actin loading control. Representative of three independent experiments. g, Trypsin-like activity in ALS iPSC-derived motor neurons on PSME3 knockdown (mean ± s.e.m. relative slope to 37 °C NT shRNA, n = 3 independent experiments). h, Immunocytochemistry of ALS iPSCs-derived motor neurons with anti-cleaved caspase-3 (red), anti-MAP2 (green) and Hoechst (blue). Scale bar: 20 µm. Graph represents the percentage of cleaved caspase-3-positive cells/total nuclei (mean ± s.e.m. of nine biological replicates, 37 °C + NT shRNA: 822 total nuclei; 37 °C + psme-3 shRNA: 669; 36 °C + NT shRNA: 803; 36 °C + psme-3 shRNA: 567). In all the experiments, cells were cultured at 37 °C and then shifted to cold temperature (36 °C) or maintained at 37 °C for 24 h before the analysis. Two-tailed Student’s t-test for paired (a,c,g) or unpaired samples (e,h). P value: *P < 0.05, **P < 0.01, ***P < 0.001, ****P < 0.0001; NS, P > 0.05. All the significant changes were also significant after correction for multiple testing by FDR (q value< 0.05). Source Data contains exact P and q values.
Source data
Prompted by these findings, we asked whether cold temperature can ameliorate disease-related neurodegeneration. ALS is characterized by the selective degeneration of motor neurons53. Accordingly, motor neurons differentiated from patient-derived induced pluripotent stem cells (iPSCs) exhibit increased cell death phenotypes54,55,56,57. Indeed, iPSC-derived motor neurons harboring the severe ALS-linked FUSP525L mutation58 had increased apoptotic rates compared with isogenic controls (FUSWT/WT) (Fig. 8e). Notably, cold temperature (36 °C) attenuated the elevated apoptotic rates of ALS motor neurons (Fig. 8e). To assess whether these effects are mediated by PA28γ/PSME3, we generated stable PSME3-shRNA ALS-iPSCs and differentiated them into motor neurons (Fig. 8f). The nervous system express the highest levels of PA28γ/PSME3 compared with other tissues32. Consistently, we found that iPSC-derived motor neurons have higher (over threefold) basal trypsin-like activity than HEK293 cells at standard temperature, whereas the other proteasome activities were decreased (Extended Data Fig. 8a-c). Although iPSC-derived motor neurons already displayed elevated trypsin-like activity at standard conditions, cold temperature could further increase this specific activity without affecting chymotrypsin-like and caspase-like activities (Fig. 8g and Extended Data Fig. 8d,e). Knockdown of PA28γ/PSME3 decreased trypsin-like activity in motor neurons at both standard and cold temperature, but the reduction was significantly stronger at cold temperature (Fig. 8g). In correlation with this decline in trypsin-like activity, knockdown of PSME3 reduced the ameliorative effects of cold temperature in the neurodegeneration phenotype of ALS motor neurons (Fig. 8h). Together, our results indicate that cold temperature prevents pathological phenotypes such as protein aggregation and neurodegeneration, a process mediated by the induction of trypsin-like activity in a PA28γ-dependent manner.
PA28γ promotes protein degradation in nucleus and cytoplasm
Because proteasomes are active in both the cytoplasm and nucleus59, we asked in which subcellular compartment PA28γ/PSME3 promotes degradation of disease-related proteins. Although PA28γ/PSME3 is mostly located in the nucleus of distinct human cell lines60,61, cumulative evidence demonstrates that PSME3 also becomes more prominent in the cytoplasm depending on the conditions and cell type62,63. Indeed, we found that the subcellular distribution varies depending on the cellular type. In the C. elegans germline and muscle, PSME-3 mostly accumulated in the nucleus (Extended Data Fig. 2d). In intestinal cells, PSME-3 was mostly present in the cytoplasm but also detected in the nucleus. Likewise, PSME-3 was present in both the soma and nucleus of C. elegans neurons, but we did not detect PSME-3 in neuronal extensions (Extended Data Fig. 2d). We observed a similar distribution of PSME3 in the soma and nucleus of human iPSC-derived motor neurons (Extended Data Fig. 9a). In contrast, PSME3 was mostly accumulated in the nucleus of HEK293 cells, although we also observed cytoplasmic PSME3 to a lesser extent (Extended Data Fig. 9b). Importantly, cold temperature did not change the subcellular distribution of PSME3 in C. elegans or human cells (Extended Data Fig. 2a–c and Extended Data Fig. 9b).
TDP-43 and FUS shuttle between the nucleus and the cytoplasm, but mostly localize in the nucleus64,65,66. However, familial ALS mutations in TDP-43 and FUS increase their cytosolic localization67,68. Accordingly, ALS-linked FUSP525L mutant variant is located in both the nucleus and cytoplasm of C. elegans and human cells, whereas wild-type FUS is essentially located in the nucleus44,47,58. Accordingly, we observed increased cytoplasmic localization of mutant FUSP525L in both human motor neurons and HEK293 cells, but a fraction of mutant FUS remained in the nucleus (Extended Data Fig. 9a,c). We found that cold temperature does not change the subcellular distribution of mutant FUSP525L in human HEK293 cells (Extended Data Fig. 9c). Likewise, cold temperature did not alter the subcellular distribution of PSME3, which remained mostly located in the nucleus (Extended Data Fig. 9b). Thus, we assessed whether cold-induced PSME3 prevents aggregation of mutant FUS by inducing its degradation in the cytoplasm or nucleus. To this end, we treated HEK293 cells with leptomycin B, an inhibitor of nuclear export69. Notably, leptomycin B treatment for 6 hours was sufficient to induce the accumulation of mutant FUSP525L in the nucleus (Extended Data Fig. 10a). Subsequently, leptomycin B treatment further increased the cold-induced degradation of mutant FUSP525L protein (Extended Data Fig. 10b). Although leptomycin B also accumulated FUSP525L in the nucleus at normal temperature (37 °C), it did not promote FUS degradation under this condition (Extended Data Fig. 10a,b). Thus, PSME3 preferentially promotes cold-induced degradation of FUSP525L in the nucleus, a process that could eventually reduce the loading of mutant FUS into the cytoplasm and subsequent aggregation. In contrast to FUS, mutant HTT is mainly cytoplasmic70 and remained in the cytoplasm upon leptomycin B treatment (Extended Data Fig. 10c). Concomitantly, leptomycin B did not further increase the cold-induced degradation of mutant HTT (Extended Data Fig. 10d). Because cold temperature was sufficient to promote HTT degradation without leptomycin B treatment (Extended Data Fig. 10d), these data suggest that even the relative low amounts of PSME3 in the cytoplasm of HEK293 cells can scavenge HTT. Thus, cold-induced PSME3 may promote degradation of aggregation-prone proteins in either the nucleus or cytoplasm, depending on the main subcellular localization of the disease-related proteins.